This review explores key hyperaccumulator species, their physiological mechanisms for metal uptake, and advancements in genetic engineering, soil amendments, and microbial-assisted strategies to enhance bioavailability and translocation.
A Review of Recent Advances in Metal Recovery through Hyperaccumulator Phytomining
Dr. Raj Shah and Michael Lotwin | Koehler Instrument Company
Abstract
Phytomining utilizes hyperaccumulator plants to extract nickel, zinc, and other valuable metals from contaminated or metal-rich soils, addressing environmental concerns associated with conventional mining such as high energy use, habitat disruption, and pollution. This review explores key hyperaccumulator species, their physiological mechanisms for metal uptake, and advancements in genetic engineering, soil amendments, and microbial-assisted strategies to enhance bioavailability and translocation. Methods for metal recovery, including bioleaching, thermal processing, and electrochemical deposition, are evaluated for efficiency and scalability. Key challenges such as biomass yield variability, site availability, and economic feasibility are discussed, alongside strategies like hybrid mining models and policy-driven incentives to facilitate commercial adoption. Emerging research in multi-metal phytomining and precision agronomy is also examined as pathways to improving extraction efficiency and expanding phytomining applications.
Introduction
Phytomining is an emerging technique utilizing hyperaccumulator plants to extract valuable metals from soil, providing an environmentally sustainable solution to traditional mining practices that typically involve intensive energy use, habitat disruption, and hazardous waste production [1]. Conventional mining methods depend on chemical extraction processes and high-temperature smelting, which result in extensive soil contamination, atmospheric pollution, and detrimental ecological impacts. In contrast, phytomining employs plants with specialized physiological mechanisms capable of accumulating metals at exceptionally high concentrations. These plants absorb metal ions via root systems, transport them through xylem-loading proteins, and compartmentalize them in vacuoles or bind them to organic ligands, significantly reducing toxicity [2]. Species notably effective for nickel phytomining include Alyssum murale, Odontarrhena chalcidica, and Phyllanthus rufuschaneyi due to their high nickel uptake efficiency, adaptability to various soil conditions, and promising scalability in phytomining applications [2].
Recent research has enhanced phytomining efficiency through genetic engineering, targeted soil amendments, and microbial-assisted metal solubilization. Genetic modifications targeting metal transport proteins, specifically ZIP, NRAMP, and HMA4, have improved the efficiency of root-to-shoot metal translocation and plant tolerance to metal stress, directly addressing challenges in maintaining plant health and maximizing metal recovery [2]. Furthermore, amendments with nitrogen and phosphorus fertilizers boost biomass production, thereby increasing metal yields per hectare and improving economic viability of phytomining operations [3]. Microbial strategies have also proven beneficial, where inoculants containing siderophore-producing bacteria such as Pseudomonas and Bacillus species significantly enhance metal solubility in soils, facilitating higher plant metal uptake and contributing to more efficient field-scale phytomining processes [2].
Despite these advancements, phytomining still faces challenges concerning consistent metal uptake rates, biomass production limitations, land availability, and efficient biomass processing methods. Metal recovery techniques including thermal processing, bioleaching, and electrochemical deposition each present their own limitations regarding energy demand, environmental footprint, and operational scalability [1]. Economic feasibility further complicates phytomining viability, as it is influenced by fluctuating metal market prices and the availability of optimal phytomining locations. This review evaluates physiological mechanisms underlying metal hyperaccumulation, examines innovations aimed at enhancing phytomining efficiency, and explores viable strategies for large-scale implementation, emphasizing sustainable recovery of metals beyond nickel and zinc.
Mechanisms of Metal Uptake and Accumulation
Hyperaccumulator Plant Physiology
Hyperaccumulator plants exhibit a unique ability to absorb and store high concentrations of heavy metals in their tissues without suffering phytotoxic effects. Unlike most plants, which actively limit metal uptake to avoid toxicity, hyperaccumulators possess specialized physiological mechanisms that enable them to accumulate nickel (Ni) at levels exceeding 1,000 mg/kg in dry biomass, often reaching concentrations several hundred times higher than those found in non-hyperaccumulating species [1].
As illustrated in Figure 1, metal uptake occurs primarily through root systems, where Ni ions are absorbed from the rhizosphere via specialized membrane transporters. Once inside the plant, Ni is translocated through the xylem in complex forms bound to organic ligands like histidine or citrate, which facilitate long-distance transport and prevent cellular toxicity [2]. Unlike metal-tolerant species that compartmentalize excess metals in root vacuoles to minimize toxicity, hyperaccumulators preferentially transport Ni ions to shoots and leaves, where they are sequestered in vacuoles or cell walls. This hyperaccumulation trait is hypothesized to serve multiple ecological functions, including herbivore deterrence and pathogen resistance, providing an evolutionary advantage in Ni-rich soils [3].
Of the 450 hyperaccumulator species, Alyssum murale, Odontarrhena chalcidica, and Phyllanthus rufuschaneyi (shown in Figure 2) demonstrate the highest Ni uptake efficiency, most rapid biomass production, and greatest adaptability to diverse soil conditions, making them ideal for Ni phytomining [4].
Metal Uptake and Transport Pathways
Ni uptake in hyperaccumulator plants is mediated by a series of specialized transporters that facilitate metal absorption and movement within plant tissues. These transporters belong primarily to the ZIP (ZRT-IRT-like protein) and NRAMP (Natural Resistance-Associated Macrophage Protein) families, which are responsible for the influx of divalent cations, like Ni²⁺, across root cell membranes [5]. The efficiency of Ni uptake is strongly influenced by soil properties such as pH, organic matter content, and the presence of competing cations like calcium (Ca²⁺) and magnesium (Mg²⁺), which can inhibit Ni availability [6].
Once nickel ions are absorbed into root cortex cells, they are moved into the xylem through xylem-loading proteins, frequently in chelated forms complexed with organic ligands like citrate and histidine, which stabilize the metal ions and prevent their precipitation within vascular tissues during transport [7]. The efficiency of this transport is commonly quantified using translocation factors, defined as the ratio of metal concentration in shoots to roots. Hyperaccumulator species frequently exhibit nickel translocation factors exceeding 10, a value considerably higher than typical non-hyperaccumulating plants, where translocation factors rarely surpass 1, emphasizing the hyperaccumulators’ distinct ability to preferentially allocate nickel to aerial biomass [7,8].
To mitigate metal toxicity, plants employ vacuolar sequestration mechanisms in leaf mesophyll cells, where Ni is compartmentalized in vacuoles to prevent interaction with essential cellular components. Studies by Mustafa et al. using X-ray fluorescence microscopy and synchrotron-based spectroscopy demonstrated that Ni presence in hyperaccumulator leaves is predominantly localized within vacuoles and cell walls, with significant enrichment in epidermal tissues (shown in Figure 3). Their findings indicate that vacuolar sequestration plays a primary role in detoxification by limiting Ni interaction with cytoplasmic components, thereby reducing oxidative stress and preventing cellular damage. Additionally, spectral analysis confirmed the association of Ni with organic acids such as citrate and histidine, further stabilizing its compartmentalization [9]. Understanding these pathways offers a foundation for enhancing phytomining efficiency through genetic engineering or soil amendment strategies.
Soil and Microbial Interactions
Ni phytomining efficiency is strongly influenced by soil chemistry and microbial activity, both of which play critical roles in determining metal bioavailability. Soil pH is a key factor, as acidic conditions (pH < 6) enhance Ni solubility, while alkaline environments promote Ni precipitation and reduce uptake efficiency [10]. Organic matter and humic substances can modify Ni availability further by forming stable complexes that facilitate or hinder root uptake, depending on the composition of the soil solution [11].
Microbial communities in the rhizosphere, particularly metal-resistant bacteria, and mycorrhizal fungi, can enhance Ni phytomining by increasing Ni solubility and facilitating root uptake. Certain plant-growth-promoting rhizobacteria (PGPR), including species of Pseudomonas and Bacillus, produce siderophores—organic molecules that bind and mobilize Ni—thereby increasing bioavailability for plant uptake [12]. Additionally, arbuscular mycorrhizal fungi (AMF) have improved plant biomass production under metal-rich conditions, as they enhance nutrient acquisition and stress tolerance [13].
Recent studies have explored the potential of bioaugmentation strategies, where Ni-solubilizing bacterial inoculants are introduced into the rhizosphere to enhance metal uptake. For example, inoculation with Pseudomonas putida strains has reportedly increased Ni accumulation in Alyssum species by up to 40%, demonstrating the potential of microbial-assisted phytomining [14]. Therefore, optimizing microbial consortia and soil amendments to further improve Ni recovery efficiency will be paramount to future research efforts.
Metal Storage and Detoxification
To tolerate the high Ni concentrations in their tissues, hyperaccumulator plants use multiple detoxification mechanisms, including vacuolar sequestration, ligand binding, and antioxidant responses. The primary storage sites for Ni in hyperaccumulators are the vacuoles of leaf epidermal and mesophyll cells, where metals are compartmentalized to prevent cytoplasmic toxicity [15]. This sequestration process is facilitated by metal-binding ligands, such as organic acids (citrate, malate) and metallothionein-like peptides, which stabilize Ni ions and prevent oxidative damage [16].
In addition to compartmentalization, hyperaccumulators employ enhanced antioxidant defense systems to mitigate the oxidative stress associated with high metal accumulation. Enzymes like superoxide dismutase (SOD), catalase (CAT), and peroxidases play a crucial role in scavenging reactive oxygen species (ROS) generated by excess metal ions [17]. Studies have shown that Ni-hyperaccumulators possess elevated levels of antioxidant enzyme activity compared to non-hyperaccumulating species, suggesting that oxidative stress management is an essential component of metal tolerance [18].
Recent advances in transcriptomic and proteomic analyses have identified key regulatory genes involved in Ni detoxification, including vacuolar transporters and stress-response proteins. For instance, García de la Torre et al. conducted a cross-species RNA-Seq analysis and identified a significant upregulation of metal tolerance proteins (MTPs) in Odontarrhena species, with MTP1 expression levels being 3.5-fold higher in hyperaccumulators compared to non-hyperaccumulators. This enhanced expression correlated with increased Ni sequestration in vacuoles, reinforcing the role of MTPs in metal compartmentalization. Their findings provide potential targets for genetic engineering approaches aimed at improving phytomining efficiency [19].
Recent Advances in Nickel Phytomining
Hyperaccumulator Plant Selection and Breeding
The selection and genetic improvement of hyperaccumulator plants are critical steps toward enhancing phytomining efficiency. Species such as Alyssum murale, Odontarrhena chalcidica, and Phyllanthus rufuschaneyi have been extensively studied for their exceptional ability to accumulate nickel (Ni) concentrations exceeding 20,000 mg/kg in shoot biomass under optimized conditions [20]. These plants possess physiological traits such as high transpiration rates and specialized metal transport proteins, allowing them to effectively extract Ni from ultramafic soils characterized by low fertility and high metal content. However, inherent variability in Ni uptake efficiency across different populations remains a significant obstacle for consistent performance in large-scale phytomining operations, highlighting the need for targeted genetic and breeding strategies.
Recent advancements in genetic engineering have provided insights into mechanisms that can significantly enhance Ni accumulation. Fasani et al. demonstrated that exposure to 100 µM NiSO₄ increased the expression level of metal transporters NRAMP4 and ZNT1 in the hyperaccumulator Noccaea caerulescens by approximately 50% compared to control conditions (shown in Figure 4) [5]. Their research involved transforming Arabidopsis thaliana, a model organism closely related to hyperaccumulators, to study transporter functions and metal accumulation processes. The authors reported that increased transporter expression not only facilitated greater Ni absorption but also enhanced metal translocation efficiency from roots to aerial tissues, a critical step in effective phytomining. Additionally, the upregulation of nicotianamine synthase (NAS), as investigated by Chaney and Baklanov, has been shown to improve metal chelation within the cytoplasm, forming stable complexes that mitigate cellular toxicity and improve metal mobility, ultimately enhancing overall Ni accumulation in plants [22].
The ability to regulate metal translocation and compartmentalization through genetic engineering is crucial for improving phytomining efficiency. Modulating the expression of these transporters could allow plants to accumulate higher metal concentrations without experiencing phytotoxicity, thereby increasing biomass yield and metal recovery. Furthermore, optimizing metal transport mechanisms through genetic modification could make phytomining more economically competitive by reducing the need for chemical amendments and increasing extraction efficiency per unit area. By integrating these molecular engineering strategies with selective breeding, researchers can further refine hyperaccumulator species for scalable and sustainable metal recovery.
Soil Amendments and Optimization Techniques
The efficiency of phytomining is largely dependent on soil conditions that influence Ni bioavailability. For instance, in ultramafic soils, only 2–5% of total Ni is bioavailable, necessitating the use of chemical, biological, and agronomic amendments to enhance plant uptake [22]. Fertilizer application is a crucial factor, as nitrogen (N2) and phosphorus (P) enhance plant biomass production and, thereby increase overall Ni extraction. However, excessive application can alter rhizosphere pH and cation exchange dynamics, reducing metal uptake. For example, a study on Alyssum murale found that a 50:50 N2/P application ratio increased Ni yield per hectare by 18%, whereas excessive N2 reduced translocation efficiency by disproportionately increasing shoot biomass relative to metal uptake [6,24].
Chelating agents have been widely studied to improve Ni solubility and root uptake. Synthetic chelators such as ethylenediaminetetraacetic acid (EDTA) can increase Ni bioavailability by up to 300%, but concerns over long-term environmental persistence have prompted research into biodegradable alternatives such as citric acid and histidine [25]. Citric acid, in particular, has been shown to increase Ni uptake in hyperaccumulators by 40% in acidic soils but is less effective in neutral conditions due to rapid microbial degradation [25].
Biological amendments, including microbial inoculants, have also shown promise in enhancing Ni uptake. Siderophore-producing Pseudomonas and Bacillus strains play a critical role in increasing Ni bioavailability by secreting low-molecular-weight organic acids such as citrate and oxalate, which chelate metal ions and prevent their precipitation in the soil. Additionally, these bacteria modify rhizosphere redox conditions, influencing metal speciation and enhancing Ni solubility for plant uptake. Rajkumar et al. demonstrated that inoculation with siderophore-producing Pseudomonas strains significantly increased Ni accumulation in Noccaea caerulescens by up to 40% under controlled conditions, highlighting their potential for large-scale phytomining applications [12]. Similarly, Visioli et al. reported that endophytic inoculants, including Bacillus strains, enhanced Ni phytoextraction in hyperaccumulators growing in serpentine soils by altering rhizosphere pH and mobilizing metal ions [14]. Arbuscular mycorrhizal fungi (AMF) is another microbial inoculant known to improve plant tolerance for metal toxicity and increase root surface area, causing as much as 25% greater Ni accumulation in Berkheya coddii and Noccaea caerulescens [13,15]. Ongoing research is exploring bioaugmentation strategies using engineered microbial consortia to maximize Ni mobilization while maintaining soil health.
Field Applications and Large-Scale Trials
Field-scale phytomining trials have provided critical insights into the scalability, economic feasibility, and agronomic challenges of Ni recovery from plants. Large-scale trials in Albania, New Caledonia, and Malaysia have demonstrated variable Ni yields ranging from 50 to 150 kg/ha per harvest cycle, depending on plant species, soil conditions, and agronomic interventions [14]. Bani et al. conducted a five-year study in which optimized agronomic conditions, including targeted fertilization with nitrogen and phosphorus, high-density planting, and soil pH adjustments, led to a Ni biomass yield of 100 kg/ha per year in Alyssum murale. Industrial-scale implementation of these conditions demonstrated economic feasibility and increasing profitability when Ni market prices exceeded $15,000 per metric ton. The estimated extraction costs were $8,500–$12,000 per metric ton, making phytomining competitive with lateritic ore processing, which incurs significant environmental costs due to acid leaching and smelting waste production [4]. Comparative studies with conventional Ni extraction methods suggest that phytomining offers a lower-carbon alternative with reduced waste generation, but improvements in biomass processing efficiency remain essential.
Despite these results, large-scale phytomining faces several logistical and agronomic challenges. Land-use constraints limit the availability of suitable sites, as phytomining requires multiple cropping cycles to extract commercially viable Ni concentrations. Thus, it is only economically competitive on marginal lands unsuitable for conventional agriculture [16]. Continuous metal extraction can also lead to soil nutrient depletion, necessitating rotational cropping with nitrogen-fixing species to maintain soil fertility [4]. Furthermore, variability in metal accumulation between plant populations necessitates further breeding and genetic selection to ensure consistent yields across multiple growing seasons.
To address the challenges in optimizing phytomining practices, recent advancements in precision agronomy and remote sensing technologies have been explored. Unmanned Aerial Vehicles (UAVs) equipped with hyperspectral imaging sensors are now utilized to monitor crop health and metal accumulation in real-time. Hyperspectral imaging captures reflectance data across multiple spectral bands, enabling precise detection of nutrient deficiencies, metal-induced stress, and overall plant physiological status [9]. This imaging technology allows for adaptive fertilization strategies to enhance metal extraction efficiency by identifying spatial variations in metal bioavailability and plant uptake [5]. Lima et al. conducted a detailed investigation on UAV-based hyperspectral imaging, demonstrating its efficacy in correlating spectral reflectance signatures with nickel accumulation in hyperaccumulators [31]. By utilizing high-resolution hyperspectral sensors, their study quantified metal-induced spectral variations in Bornmuellera emarginata and Lupinus albus, confirming that reflectance indices could reliably predict nickel concentrations in plant tissues. The researchers applied machine learning models, including partial least squares regression (PLSR), to validate spectral data against ground-truth measurements of nickel accumulation, achieving a predictive accuracy exceeding 85%. These findings demonstrate the potential of remote sensing technologies for optimizing large-scale phytomining operations, enabling real-time monitoring of metal uptake and facilitating precision agronomic interventions [31].
Beyond remote sensing, soil amendments such as biochar have been investigated for their role in enhancing phytomining efficiency. Chaney and Baklanov demonstrated that biochar amendments significantly enhance nickel bioavailability in hyperaccumulator cropping systems by modifying soil physicochemical properties, including cation exchange capacity and pH buffering [31]. Their research highlighted that biochar derived from lignocellulosic biomass improves nickel solubilization by increasing organic acid exudation in the rhizosphere, thereby promoting root-metal interactions. Additionally, Rajkumar et al. reported that biochar supplementation, when combined with microbial inoculants such as siderophore-producing Pseudomonas and Bacillus strains, further amplified nickel uptake efficiency by up to 40% through enhanced metal chelation and redox alterations [12]. These studies collectively emphasize the role of biochar in phytomining optimization, particularly in serpentine soils where nickel bioavailability is often constrained by mineralogical binding [12, 23].
Biomass Processing and Metal Recovery
The final step in the phytomining process is Ni extraction from harvested biomass, which determines overall efficiency and environmental impact. Of the current processing techniques, thermal incineration is the most established, producing Ni-rich ash with metal concentrations 10–30 times higher than that in raw biomass; however, combustion releases CO₂ and contributes significantly to air pollution [22]. As an alternative, pyrolysis conducted at temperatures between 450–600°C can increase Ni recovery efficiency while significantly reducing carbon emissions compared to traditional incineration. This enhanced efficiency is primarily due to the selective conversion of biomass into biochar, concentrating metals into a stable, easily recoverable form and minimizing the loss of volatile metal compounds [31].
Bouman et al. reported that bioleaching, a microbial-assisted metal recovery process, utilizes acidophilic bacteria such as Acidithiobacillus ferrooxidans and Leptospirillum ferrooxidans to enzymatically extract Ni from biomass. Their study demonstrated that Ni recovery rates reached up to 85% within 10 days, highlighting the potential of bioleaching as a cost-effective alternative to conventional smelting techniques, which are energy-intensive and produce significant carbon emissions [20]. However, the scalability of bioleaching remains a challenge due to fluctuations in microbial activity influenced by environmental factors, including pH variation, temperature instability, and nutrient availability, necessitating further optimization to enhance process efficiency in large-scale applications.
Electrochemical recovery has emerged as a highly efficient extraction alternative, leveraging electrodeposition techniques to selectively extract Ni from plant-derived solutions. Experimental results using graphite and platinum electrodes have achieved Ni recovery efficiencies exceeding 90% under optimized voltage and electrolyte conditions [21]. This method eliminates the need for high-temperature smelting, reducing energy consumption and operational costs. Additionally, bioleaching has been explored as a complementary process, utilizing acidophilic bacteria such as Acidithiobacillus ferrooxidans and Leptospirillum ferrooxidans to enzymatically extract Ni from biomass. Bouman et al. demonstrated that under optimized conditions, bioleaching achieved Ni recovery rates of up to 85% within 10 days, significantly lowering processing costs [20]. However, bioleaching scalability remains a challenge due to variability in microbial activity under fluctuating temperature and pH conditions. Bouman’s study also showed that pH levels below 2.5 optimize Ni solubilization, but extreme acidity may inhibit bacterial growth and require controlled bioreactor conditions for maximum efficiency [20].
Phytomining for Zinc and Other Metals
Zinc-Hyperaccumulating Plants and Mechanisms
Zinc (Zn) contamination is a widespread environmental issue due to industrial activities, mining, and agricultural practices, posing significant risks to ecosystems and human health; phytomining using Zn-hyperaccumulator plants offers a promising solution for remediating these contaminated areas. Hyperaccumulator species such as Thlaspi caerulescens, Noccaea caerulescens, and Arabidopsis halleri have been extensively studied for their remarkable ability to accumulate Zn at concentrations exceeding 10,000 mg/kg in shoot tissues under field conditions [2,8]. Unlike Ni hyperaccumulators, which typically store metals in vacuoles, Zn hyperaccumulators predominantly utilize plasma membrane transporters, including ZNT1, ZNT5, and HMA4, to mediate Zn uptake, efficient translocation, and cellular detoxification [5]. Understanding these mechanisms is crucial for optimizing Zn phytomining strategies and ensuring effective remediation of Zn-contaminated environments.
A key distinction between Ni and Zn hyperaccumulation lies in their metal storage mechanisms. While Ni is often chelated with organic acids such as citrate or histidine, Zn is typically complexed with phytochelatins, metallothioneins, and sulfate [8,16]. This difference in metal handling directly influences plant physiology and has critical implications for phytomining efficiency. Specifically, the complexation of Zn with phytochelatins and metallothioneins allows plants to sustain higher Zn concentrations in aerial tissues without experiencing phytotoxicity, potentially making Zn hyperaccumulators more effective candidates for phytomining operations by facilitating efficient biomass harvesting and metal recovery. However, this complexation process might also demand additional considerations for optimizing extraction procedures due to the stronger metal-ligand interactions involved [8,16].
Additionally, Zn hyperaccumulators exhibit differential responses to soil metal concentrations. Thlaspi caerulescens exhibits inducible Zn uptake, wherein plants increase Zn influx only in Zn-enriched soils, while Arabidopsis halleri maintains a constitutive Zn uptake system, making it a more robust candidate for Zn phytomining in variable environments [5]. These physiological adaptations highlight the need for species-specific strategies in Zn phytomining applications.
Soil Optimization for Zinc Phytomining
Soil characteristics significantly influence Zn bioavailability and phytomining efficiency. The solubility of Zn is strongly pH-dependent, with optimal uptake in soils with a pH range of 5.5–6.5, where Zn exists as bioavailable Zn²⁺ ions. In contrast, alkaline conditions promote Zn precipitation as ZnCO₃ and Zn(OH)₂, reducing plant uptake [10].
Organic matter amendments, such as compost or biochar, can significantly enhance zinc (Zn) solubility and phytomining efficiency by altering soil properties like pH, cation exchange capacity, and metal chelation dynamics. Park et al. (2011) demonstrated that organic amendments, particularly compost and biochar, effectively increased Zn bioavailability by modifying rhizosphere conditions and promoting metal chelation through the release of organic acids. These amendments not only mobilize Zn ions from less available soil fractions but also stimulate microbial activity, further improving nutrient cycling and metal solubility [11].
Biological strategies involving microbial inoculants have also shown substantial potential. Rajkumar et al. (2010) conducted controlled experiments using siderophore-producing Pseudomonas strains with Thlaspi caerulescens, demonstrating that inoculation significantly improved Zn uptake by up to 40%. The siderophores produced by these bacteria effectively chelate Zn ions, preventing their precipitation in the soil and facilitating plant uptake. This study employed carefully controlled pot trials with standardized soil and bacterial inoculant conditions, ensuring accurate assessment of microbial effectiveness in enhancing Zn phytoextraction. These findings highlight the feasibility of microbial-assisted phytomining as a scalable and environmentally friendly alternative to conventional chemical amendments [12].
Chelating agents such as EDTA, DTPA, and citrate have also been investigated for Zn phytomining. While EDTA significantly enhances Zn solubility, its long persistence in soils raises concerns about groundwater contamination. More environmentally benign chelators, such as citric acid and histidine, have been shown to significantly enhance zinc (Zn) uptake efficiency while reducing environmental risks. For instance, in wheat, citric acid increased Zn root uptake by approximately 3.5 times, and histidine by about 9 times, compared to EDTA treatments [25] Additionally, arbuscular mycorrhizal fungi (AMF) improve Zn uptake by increasing root surface area and metal transport efficiency, making them a promising addition to soil management strategies for Zn phytomining [13]. Soil optimization strategies, encompassing organic amendments, microbial inoculants, and targeted use of chelating agents, are therefore crucial to enhancing zinc bioavailability and uptake efficiency. Effective management of soil properties can significantly improve phytomining outcomes, highlighting the importance of developing tailored agronomic practices for sustainable and economically viable metal recovery.
Comparative Economic and Environmental Feasibility
Although Zn and Ni phytomining share similar methodologies, key economic and environmental factors vary their feasibilities. Ni phytomining has demonstrated commercial viability due to the high market value of Ni ($15,000–$20,000 per metric ton) and its relatively low energy processing costs. In contrast, Zn phytomining faces economic challenges as Zn prices are lower (~$3,000 per metric ton) and global availability of Zn from conventional mining sources is high[22].
Despite this disparity, Zn phytomining offers unique advantages in contaminated land rehabilitation. Unlike Ni, which is predominantly mined from ultramafic soils, Zn contamination is widespread due to industrial pollution, smelting activities, and mine tailings [10]. Thus, Zn phytomining acts as a dual-purpose strategy— recovering Zn while remediating contaminated soils. Hipfinger et al. reported that large-scale Zn phytomining trials yielded up to 30 kg Zn per hectare per harvest cycle, demonstrating its potential as a remediation approach in Zn-contaminated regions (shown in Figure 5). Their study emphasized the importance of optimizing fertilization regimes to enhance Zn bioavailability and uptake efficiency, highlighting key agronomic factors influencing metal recover [24].
One emergent field of research involves co-mining multiple metals from the same site. Some hyperaccumulators, such as Noccaea caerulescens, can accumulate both Zn and Ni, presenting opportunities for multi-metal recovery systems [5,14]. By optimizing soil conditions to favor the uptake of multiple target metals, phytomining operations could achieve greater profitability and resource efficiency. Given these factors, Zn phytomining holds considerable promise due to its advantages in environmental remediation, metal recovery from polluted sites, and potential integration into multi-metal extraction systems. Despite economic constraints, the strategic value of Zn as both an industrial resource and a driver of sustainable land rehabilitation shows the importance of advancing research into optimized agronomic and processing approaches.
Expanding Phytomining to Other Metals
Beyond Zn and Ni, phytomining is viable for other high-value metals, including cobalt (Co), manganese (Mn), and rare earth elements (REEs). Among these, cobalt phytomining is particularly promising due to cobalt's high economic value and important role in battery production and renewable energy technologies [23]. Hyperaccumulator species such as Crotalaria cobalticola and Haumaniastrum robertii have demonstrated exceptional potential, accumulating up to 10,000 mg/kg of cobalt in shoot tissues [1,20]. However, cobalt phytomining faces significant challenges, primarily related to the limited bioavailability of cobalt in lateritic soils. Addressing these challenges has driven research into soil amendment strategies, notably the use of chelators and microbial-assisted mobilization techniques, which could enhance cobalt solubilization and bioavailability, thereby improving the efficiency and scalability of cobalt phytomining operations.
Rare earth elements (REEs), including neodymium (Nd), dysprosium (Dy), and yttrium (Y), represent another critical and economically valuable target for phytomining due to their widespread applications in electronics, renewable energy technologies, and defense systems. Despite the rarity of natural REE hyperaccumulators, some promising candidates have emerged within the Euphorbiaceae and Fabaceae families, notably for cerium (Ce) and lanthanum (La) uptake from lateritic soils [1,23]. Given the high market demand and value of certain REEs (~$100,000 per metric ton for selected elements), phytomining provides a sustainable alternative to conventional extraction methods, such as ion-adsorption clay mining, which carry significant environmental impacts. Future research should prioritize developing targeted genetic, biological, and chemical enhancements to optimize plant performance and metal recovery methods, with particular attention to integrated, multi-metal recovery systems that could improve the economic feasibility and environmental sustainability of phytomining for cobalt and REEs.
Challenges and Future Works
While phytomining presents a promising alternative to traditional mining, several challenges must be addressed to optimize efficiency, scalability, and economic viability. Current phytomining techniques are limited by metal uptake rates, biomass yield, and metal recovery efficiency, all of which hinder widespread adoption. A significant limitation is the low accumulation rate of metals per hectare, necessitating multiple harvest cycles to obtain commercially viable quantities. Additionally, metal uptake by plants is influenced by soil composition, microbial activity, and environmental conditions, resulting in variability of metal recovery across different sites. Furthermore, the extended cropping periods required by hyperaccumulators delay metal extraction, making phytomining less attractive than conventional mining, which operates on significantly shorter timescales [26].
However, advancements in genetic modification and synthetic biology offer potential solutions to overcome these challenges. Engineering hyperaccumulator species with enhanced metal uptake pathways could significantly improve phytomining efficiency. For instance, the overexpression of metal transporters such as HMA4 and IRT1 has successfully increased zinc and iron translocation in model plants, suggesting similar approaches could enhance nickel and cobalt uptake [27]. Additionally, researchers are exploring CRISPR-based gene editing techniques to optimize root exudate production, thereby improving metal solubility in the rhizosphere and enhancing bioavailability [28]. Synthetic biology approaches are also being investigated, particularly the development of metal-binding peptides to increase metal storage capacity in plant vacuoles and mitigate metal toxicity [29].
Another promising avenue of future research is the development of multi-metal recovery systems, where phytomining could simultaneously extract nickel, cobalt, zinc, and rare earth elements. Certain hyperaccumulator species, such as Noccaea caerulescens, naturally accumulate multiple metals, though extraction efficiency varies with soil conditions and specific plant metabolic pathways. Recent research has indicated that co-cropping strategies, in which various hyperaccumulators are planted together, could optimize the uptake of distinct metals from a single site [30]. Moreover, advancements in bioleaching and electrochemical metal recovery techniques could further improve metal extraction efficiency from plant biomass, potentially reducing processing costs and enhancing phytomining's economic competitiveness [31].
Despite these advancements, scalability remains a significant obstacle for phytomining. The extensive land requirements necessary for commercial-scale phytomining could conflict with existing agricultural practices and conservation efforts, necessitating careful and strategic land-use planning. Additionally, fluctuations in global metal prices could affect the financial viability of phytomining projects, underscoring the importance of developing hybrid mining models that integrate phytomining with conventional extraction methods [32].
Conclusion
Phytomining presents a sustainable method for metal recovery, leveraging hyperaccumulator plants to extract nickel, zinc, and other valuable metals from the soil. The physiological mechanisms of metal uptake, the role of genetic modifications in enhancing translocation and tolerance, and soil amendments that improve metal bioavailability were all addressed and examined. While large-scale trials have demonstrated its feasibility, limitations in biomass yield, extraction efficiency, and economic competitiveness remain. Advances in genetic engineering, microbial-assisted uptake, and electrochemical recovery offer potential improvements, while hybrid models integrating phytomining with conventional extraction could enhance scalability. Ultimately, further research is needed to optimize plant-metal interactions, refine processing methods, and establish economic frameworks to support widespread implementation.
Dr. Raj Shah is a Director at Koehler Instrument Company in New York, Holtsville, NY. He is an elected Fellow by his peers at IChemE, CMI, STLE, AIC, NLGI, INSTMC, AOCS, Institute of Physics, The Energy Institute and The Royal Society of Chemistry. An ASTM Eagle award recipient, Dr. Shah recently coedited the bestseller, “Fuels and Lubricants handbook”, details of which are available at ASTM’s Long-Awaited Fuels and Lubricants Handbook 2nd Edition Now Available (https://bit.ly/3u2e6GY). He earned his doctorate in Chemical Engineering from The Pennsylvania State University and is a Fellow from The Chartered Management Institute, London. Dr. Shah is also a Chartered Scientist with the Science Council, a Chartered Petroleum Engineer with the Energy Institute and a Chartered Engineer with the Engineering council, UK. Dr. Shah was recently granted the honorific of “Eminent engineer” with Tau beta Pi, the largest engineering society in the USA. He is on the Advisory board of directors at Farmingdale university (Mechanical Technology) , Auburn Univ ( Tribology ), SUNY, Farmingdale, (Engineering Management) and State university of NY, Stony Brook ( Chemical engineering/ Material Science and engineering). An Adjunct Professor at the State University of New York, Stony Brook, in the Department of Material Science and Chemical engineering, Raj also has approximately 700 publications and has been active in the energy industry for over 3 decades. More information on Raj can be found at https://bit.ly/3QvfaLX
Contact: rshah@koehlerinstrument.com
Mr. Michael Lotwin is part of a thriving internship program at Koehler Instrument company in Holtsville, and is a student of Chemical Engineering at Stony Brook University, Long Island, NY where Dr. Shah is chair of the external advisory board of directors.
References
[1] van der Ent, A., Baker, A. J. M., Reeves, R. D., Pollard, A. J., & Schat, H. (2013). Hyperaccumulators of metal and metalloid trace elements: Facts and fiction. Plant and Soil, 362(1–2), 319–334. https://doi.org/10.1007/s11104-012-1287-3
[2] Kozhevnikova, A. D., Seregin, I. V., Verweij, R., & Schat, H. (2014). Histidine promotes the loading of nickel and zinc, but not of cadmium, into the xylem in Noccaea caerulescens. Plant Signaling & Behavior, 9(2), e27887. https://doi.org/10.4161/psb.29580
[3] Boyd, R. S. (2012). Plant defense using toxic inorganic ions: Conceptual models of the defensive enhancement and joint effects hypotheses. Plant Science, 195, 88–95. https://doi.org/10.1016/j.plantsci.2012.06.012
[4] Bani, A., Echevarria, G., Sulçe, S., & Morel, J. L. (2015). Improving the agronomy of Alyssum murale for extensive phytomining: A five-year field study. International Journal of Phytoremediation, 17(1–6), 117–127. https://doi.org/10.1080/15226514.2013.862204
[5] Fasani, E., DalCorso, G., Zorzi, G., Agrimonti, C., Fragni, R., Visioli, G., & Furini, A. (2021). Overexpression of ZNT1 and NRAMP4 from the Ni hyperaccumulator Noccaea caerulescens population Monte Prinzera in Arabidopsis thaliana perturbs Fe, Mn, and Ni accumulation. International Journal of Molecular Sciences, 22(21), 11896. https://doi.org/10.3390/ijms222111896
[6] Chaney, R. L., Chen, K. Y., Li, Y. M., Angle, J. S., & Baker, A. J. M. (2008). Effects of calcium on nickel tolerance and accumulation in Alyssum species and cabbage. Plant and Soil, 311(1–2), 131–140. https://doi.org/10.1007/s11104-008-9656-9
[7] van der Pas, L., & Ingle, R. A. (2019). Towards an understanding of the molecular basis of nickel hyperaccumulation in plants. Plants, 8(1), 11. https://doi.org/10.3390/plants8010011
[8] Verbruggen, N., Hermans, C., & Schat, H. (2009). Molecular mechanisms of metal hyperaccumulation in plants. New Phytologist, 181(4), 759–776. https://doi.org/10.1111/j.1469-8137.2008.02748.x
[9] Mustafa, A., Zulfiqar, U., Mumtaz, M. Z., Radziemska, M., Haider, F. U., Holátko, J., Hammershmidť, T., Naveed, M., Ali, H., Kintl, A., Saeed, Q., Kucerík, J., & Brtnický, M. (2023). Nickel (Ni) phytotoxicity and detoxification mechanisms: A review. Chemosphere, 328, 138574. https://doi.org/10.1016/j.chemosphere.2023.138574
[10] Zhong, X., Chen, Z., Li, Y., Ding, K., Liu, W., Liu, Y., & Qiu, R. (2020). Factors influencing heavy metal availability and risk assessment of soils at typical metal mines in Eastern China. Journal of Hazardous Materials, 400, 123289. https://doi.org/10.1016/j.jhazmat.2020.123289
[11] Park, J. H., Lamb, D., Paneerselvam, P., Choppala, G., Bolan, N., & Chung, J. W. (2011). Role of organic amendments on heavy metal immobilization in soil. Journal of Hazardous Materials, 185(2–3), 549–574. https://doi.org/10.1016/j.jhazmat.2010.09.082
[12] Rajkumar, M., Ae, N., Prasad, M. N. V., & Freitas, H. (2010). Potential of siderophore-producing bacteria for improving heavy metal phytoextraction. Trends in Biotechnology, 28(3), 142–149. https://doi.org/10.1016/j.tibtech.2009.12.002
[13] Orłowska, E., Przybyłowicz, W., Orlowski, D., Turnau, K., & Mesjasz-Przybyłowicz, J. (2011). The effect of arbuscular mycorrhiza on the growth and elemental composition of the Ni-hyperaccumulating plant Berkheya coddii. Environmental Pollution, 159(12), 3730–3738. https://doi.org/10.1016/j.envpol.2011.07.008
[14] Visioli, G., Vamerali, T., Mattarozzi, M., Dramis, L., & Sanangelantoni, A. M. (2015). Combined endophytic inoculants enhance nickel phytoextraction from serpentine soil in the hyperaccumulator Noccaea caerulescens. Frontiers in Plant Science, 6, 638. https://doi.org/10.3389/fpls.2015.00638
[15] Marshall, A. T. (2004). Sub-cellular localization of Ni in the hyperaccumulator Hybanthus floribundus (Lindley) F. Muell. Plant, Cell & Environment, 27(6), 705–716. https://doi.org/10.1111/j.0016-8025.2003.01170.x
[16] Leitenmaier, B., & Küpper, H. (2013). Compartmentation and complexation of metals in hyperaccumulator plants. Frontiers in Plant Science, 4, 374. https://doi.org/10.3389/fpls.2013.00374
[17] Soleymanifar, S., Ehsanpour, A. A., Ghasemi, R., & Schat, H. (2023). The effect of L-histidine on nickel translocation and antioxidant enzyme activities in hyperaccumulator (Odontarrhena inflata) and non-accumulator (Aurinia saxatilis) plants. Plant and Soil, 491, 1–18. https://doi.org/10.1007/s11104-023-06340-9
[18] Boominathan, R., & Doran, P. M. (2002). Ni-induced oxidative stress in roots of the Ni hyperaccumulator Alyssum bertolonii. New Phytologist, 156(2), 205–215. https://doi.org/10.1046/j.1469-8137.2002.00506.x
[19] García de la Torre, V. S., Majorel-Loulergue, C., Rigaill, G. J., Alfonso-González, D., Soubigou-Taconnat, L., Pillon, Y., … & Merlot, S. (2021). Wide cross-species RNA-Seq comparison reveals convergent molecular mechanisms involved in nickel hyperaccumulation across dicotyledons. New Phytologist, 229(2), 994–1006. https://doi.org/10.1111/nph.16775
[20] Bouman, R. W., van der Ent, A., & van Welzen, P. C. (2018). Phyllanthus rufuschaneyi: A new nickel hyperaccumulator from Sabah (Borneo Island) with potential for tropical agromining. Botanical Studies, 59, 9. https://doi.org/10.1186/s40529-018-0225-y
[21] Nishida, S., Tanikawa, R., Ishida, S., Yoshida, J., Mizuno, T., Nakanishi, H., & Furuta, N. (2020). Elevated expression of vacuolar nickel transporter gene IREG2 is associated with reduced root-to-shoot nickel translocation in Noccaea japonica. Frontiers in Plant Science, 11, 610. https://doi.org/10.3389/fpls.2020.00610
[22] Chaney, R. L., & Baklanov, I. A. (2017). Phytomining for nickel: Economic and technological prospects. In J. C. Sanchez-Hernandez (Ed.), Advances in Botanical Research (Vol. 83, pp. 223–256). Elsevier. https://doi.org/10.1016/bs.abr.2016.12.006
[23] Chowdhury, Mohammed Omar Sahed, and Deniz Talan. “From Waste to Wealth: A Circular Economy Approach to the Sustainable Recovery of Rare Earth Elements and Battery Metals From Mine Tailings.” Separations, vol. 12, no. 2, Feb. 2025, p. 52. https://doi.org/10.3390/separations12020052.
[24] Hipfinger, C., Rosenkranz, T., Thüringer, J., & Puschenreiter, M. (2021). Fertilization regimes affecting nickel phytomining efficiency on a serpentine soil in the temperate climate zone. International Journal of Phytoremediation, 23(4), 407–414. https://doi.org/10.1080/15226514.2020.1820446
[25] Gramlich, Anja, et al. “Availability of Zinc and the Ligands Citrate and Histidine to Wheat: Does Uptake of Entire Complexes Play a Role?” Journal of Agricultural and Food Chemistry, vol. 61, no. 44, Oct. 2013, pp. 10409–17. https://doi.org/10.1021/jf401117d.
[26] Ali, H., Khan, E., & Sajad, M. A. (2013). Phytoremediation of heavy metals—Concepts and applications. Chemosphere, 91(7), 869–881. https://doi.org/10.1016/j.chemosphere.2013.01.075
[27] Shanmugam, Varanavasiappan, et al. “Differential Expression and Regulation of Iron‐regulated Metal Transporters in Arabidopsis Halleri and Arabidopsis Thaliana – the Role in Zinc Tolerance.” New Phytologist, vol. 190, no. 1, Jan. 2011, pp. 125–37. https://doi.org/10.1111/j.1469-8137.2010.03606.x.
[28] Basharat, Zarrin, et al. “Genome Editing Weds CRISPR: What Is in It for Phytoremediation?” Plants, vol. 7, no. 3, June 2018, p. 51. https://doi.org/10.3390/plants7030051.
[29] Clemens, Stephan, et al. “Tolerance to Toxic Metals by a Gene Family of Phytochelatin Synthases From Plants and Yeast.” The EMBO Journal, vol. 18, no. 12, June 1999, pp. 3325–33. https://doi.org/10.1093/emboj/18.12.3325.
[30] Lima, Luiz, et al. “Co-cropping of Bornmuellera Emarginata , Lupinus Albus and Imperata Cylindrica : A Study of Metal Uptake Interactions and Nickel Phytoextraction Efficiency.” International Journal of Phytoremediation, Jan. 2025, pp. 1–10. https://doi.org/10.1080/15226514.2025.2456098.
[31] Joshi, Khyati, et al. “Strategies for Hydrocarbon Removal and Bioleaching-Driven Metal Recovery From Oil Sand Tailings.” Minerals, vol. 14, no. 11, Oct. 2024, p. 1093. https://doi.org/10.3390/min14111093.
[32] Akinbile, Babatunde Joseph, and Charles Mbohwa. “Incorporating Hyperaccumulating Plants in Phytomining, Remediation and Resource Recovery: Recent Trends in the African Region – a Review.” RSC Sustainability, Jan. 2025, https://doi.org/10.1039/d5su00021a.
[33]Van Der Pas, Llewelyn, and Robert A. Ingle. “Towards an Understanding of the Molecular Basis of Nickel Hyperaccumulation in Plants.” Plants, vol. 8, no. 1, Jan. 2019, p. 11. https://doi.org/10.3390/plants8010011.
[34] Alyssum Murale Profile – California Invasive Plant Council. www.cal-ipc.org/plants/profile/alyssum-murale-profile.
[35] “Odontarrhena Chalcidica (Janka) Španiel, Al-Shehbaz, D.A.German and Marhold | Plants of the World Online | Kew Science.” Plants of the World Online, powo.science.kew.org/taxon/urn%3Alsid%3Aipni.org%3Anames%3A77151986-1/images.
[36] Pskhun. [Botany • 2018] Phyllanthus Rufuschaneyi (Phyllanthaceae) • a New Nickel Hyperaccumulator From Sabah (Borneo Island) With Potential for Tropical Agromining. novataxa.blogspot.com/2018/05/phyllanthus-rufuschaneyi.html.
The content & opinions in this article are the author’s and do not necessarily represent the views of AltEnergyMag
Comments (0)
This post does not have any comments. Be the first to leave a comment below.
Featured Product
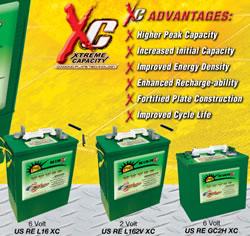