There is a growing effort toward establishing a high-tech energy-generating system that is fundamentally inspired by nature itself: artificial photosynthesis.
2021 Top Article - Artificial Photosynthesis as a Renewable Energy Source
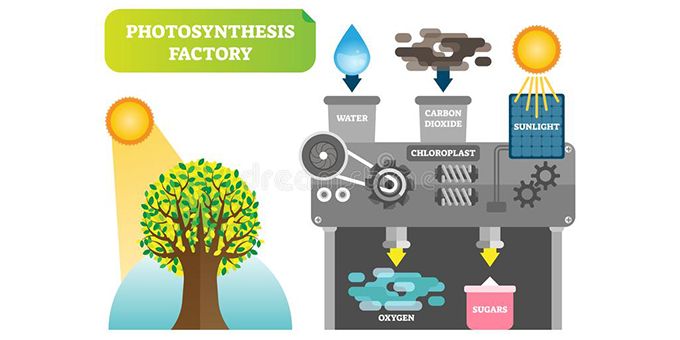
Dr. Raj Shah, Ms. Eliana Matsil, Ms. Gabrielle Massoud | Koehler Instrument Company
The urgency of realizing a clean substitute to our fossil fuel energy supply is increasing exponentially. The study of artificial photosynthesis as a renewable energy source has been carried out for decades. This approach uses biomimetic techniques to replicate the process of natural photosynthesis, which uses abundant resources of sunlight, water, and carbon dioxide to produce oxygen and energy-rich carbohydrates. By replicating this operation, researchers are able to design systems which utilize these natural resources to convert solar energy into chemical energy and store it in the bonds of a fuel. Fuels that are able to be produced by artificial photosynthesis include hydrocarbons such as formic acid (HCOOH), methanol (CH3OH), carbon monoxide (CO), and methane (CH4), or pure hydrogen fuel. The chemical processes involved in artificial photosynthesis include the splitting of water into oxygen and hydrogen, or the reduction of carbon dioxide into various hydrocarbons. These processes are accomplished by a handful of device designs, including photoelectrochemical cells or photovoltaic-coupled electrolysers. Each function is driven by energy extracted from sunlight photons as well as suitable catalysts. There is also a common method of combining advantageous components from both natural photosynthesis and artificial photosynthesis to create a semi-artificial photosynthesis system, involving the incorporation of enzymes or even whole-cell applications to synthetic devices. However, there are several limitations to the advancement of this field centered around the inability to establish a system that demonstrates the important characteristics of cost-effectiveness, long-term durability, and excellent efficiency. Nonetheless, the search for suitable materials continues as researchers are eager to institute a viable device that is ready for industrial application. Large scale employment of artificial photosynthesis can potentially provide society with renewable and storable energy in the form of valuable fuels. Hydrocarbons produced can act as substitutes for fossil fuels, and pure hydrogen can be used as a fuel as well or be channeled into a fuel cell to generate electricity. Artificial photosynthesis devices can also function as atmospheric cleansers, extracting excess carbon dioxide from the environment, and releasing oxygen back into it. Although there is a long way to go, a society powered by energy generated from artificial photosynthesis is desirable, and the endeavor to this date shows good promise.
1. Introduction
As society advances, the need to develop a renewable source of energy that is alternative to our harmful standards is ever so present. The use of conventional fossil fuels continues to both deplete natural resources as well as emit greenhouse gases which hinder the safety of our environment. Over the years, scientists have explored numerous substitutes that could contribute toward lessening our dependence on fossil fuels, which make up about 80% of the world’s energy supply [1]. Hence, there is a growing effort toward establishing a high-tech energy-generating system which is fundamentally inspired by nature itself. This concept is called artificial photosynthesis; it is designed to mimic the biological reactions that take place within natural plants, algae, and some bacteria to essentially produce their own energy which they store in chemical bonds [2].
The sun provides enough energy on the surface of the earth every thirty minutes to supply humanity’s energy demands for an entire year [3]. Unlike fossil fuels, which are unevenly distributed across the world, often causing political tension or availability issues [1], sunlight is enormously available and geographically dispersed [4]. It is argued by many that the only source strong enough to power society in the long term is solar energy [5]. In other words, the sun is our only hope.
Natural photosynthesis is solely responsible for all the energy that is burned in the world. All the energy circulated among the biological community roots from photosynthesis, which takes place in the organisms at the bottom of the food chain. Despite this, photosynthesis is also accountable for the energy stored in fossil fuels such as coal and petroleum. With this in mind, it theoretically makes sense to try and derive useful energy directly from a biomimetic approach to this natural process.
The concept of artificial photosynthesis is similar to that of the solar panels you may spot on the top of roofs or arrayed across fields. However, the photovoltaic cells found in solar panels are designed to harness solar energy and convert it into electricity for direct application. While this can be useful, the electricity produced by solar panels is limited by its dependence on weather and time [2], which is limiting by the fact that it cannot be suitably stored by batteries at this point [6]. In contrast, although artificial photosynthesis devices also rely on sun exposure, the semiconductors can absorb solar energy and store it in the chemical bonds of a fuel for later use [2, 7]. In this way, energy is being stored densely and cheaply in the form of a fuel, as opposed to the expensive and unsustainable option of battery storage [4]. This idea is emphasized in figure 1, which visualizes the difference in storage capabilities between batteries and fuels.
Figure 1. Volumetric and gravimetric energy density of various energy storage systems. [4]
Aside from the storability aspect, artificial photosynthesis is also more environmentally attractive than solar panels, for it could potentially aid in absorbing excess CO2 from the atmosphere, as well as releasing helpful oxygen back into the environment [7]. Therefore, this system has prospect to be revolutionary in the world of solar power.
In July 2020, the U.S. Department of Energy (DOE) announced a 5-year funding plan of $100 million toward research on artificial photosynthesis. The organization hopes to place America at the forefront of this field, one that is known to be of great challenge, but also great promise. The research will focus on establishing low-cost, industrially-compatible techniques of converting sunlight into usable energy at maximum efficiency [8]. In doing this, scientists have the opportunity to demonstrate a renewable system from which they can derive essential products such as liquid fuels [2]. The $100 million investment represents the country’s commitment to investigating artificial photosynthesis, for this technology has the potential to be groundbreaking in relieving the world’s current energy predicament.
2. Background
2.1. Natural Photosynthesis
To break it down, natural photosynthesis occurs within two general parts, photosystem II and photosystem I. Photosystem II is responsible for the absorption of light and its conversion into chemical energy [11]. As CO2 is absorbed through the stomata, which are openings located on the leaves [9], a collection of pigment molecules like chlorophyl called the antenna system absorbs and harvests sunlight and transfers the light energy to what is called the reaction center during the light-dependent reaction, which takes place in the thylakoid of the chloroplast [10]. Here, energy from light excites the chlorophyll molecules causing them to release an electron which travels through an electron transport chain, where ATP (adenosine triphosphate) and NADPH (nicotinamide adenine dinucleotide phosphate) are generated [12]. An electron from water fills the “electron hole” in the chlorophyll pigment releasing oxygen, often called the worlds most valuable byproduct. Driving the system all the while is a complex array of enzymes such as the photosystems themselves, as well as hydrogenases which interact with hydrogen derived from water molecules [13].
The electron arrives at Photosystem I, where the light-independent reaction takes place, which is also referred to as the dark reaction or the Calvin cycle. This occurs in the leaf’s stroma. During the Calvin cycle, water and catalysts are used to drive reactions that use the chemical energy derived from the first reaction to transform carbon atoms from CO2 into organic molecules [10]. The reduction of CO2 into carbohydrates ultimately produces glucose, as seen in this chemical formula:
6H2O + 6CO2 + (sunlight) → C6H12O6 + 6O2 [9]
Thus, natural photosynthesis is ingeniously designed by evolution to provide organisms with a complex means of producing their own food by converting solar energy into chemical energy stored in the bonds of a carbohydrate. The entire process is schematically illustrated in figure 2.
Figure 2. Schematic representation of the oxygenic photosynthesis process. [11]
2.2. Artificial Photosynthesis General Concept
The concept of artificial photosynthesis is to imitate this fundamental process that occurs within natural organisms and manipulate it to fit our societal needs. The term can refer to any scheme which captures and stores solar energy in the chemical bonds of a fuel. Hence, rather than producing glucose, valuable fuels such as hydrogen or methanol are created [14]. The general goal behind this effort is to establish a way of making energy renewable, reliable, and storable without impacting the environment in a negative way. Although the notion has not been accomplished in an industrial sense, there have been many notable successes on the lab scale [15].
2.2.1 Hydrogen Fuel
Artificial photosynthesis can produce two types of fuel: hydrocarbons such as methanol and formic acid, or simply pure hydrogen. Hydrogen is emerging as a clean fuel option that can either be consumed in a fuel cell or used directly as a liquid fuel itself [2]. It can be utilized for transportation (for example, in certain cars), to power homes, or other applications to substitute fossil fuels. When channeled into a fuel cell, it can also generate electricity. This type of fuel is produced using a number of methods, artificial photosynthesis appearing as one of them. Other techniques include thermal processes, electrolysis, biological processes, or other solar-driven systems [16].
2.2.2 Essential Components
There are three major components to an artificial photosynthesis device for which there is a need to develop: light capture and electron transport, water splitting (into hydrogen and oxygen), and the reduction of carbon dioxide [6]. Researchers have established a handful of systems that are able to carry out these important processes [2]. The components of these systems are synthetically designed to function as corresponding elements in the reaction centers of natural photosynthesis, such as pigment molecules and electron transport chains.
2.2.3 Limitations and Challenges
When attempting to replicate the functionality of autotrophs in a practical way, researchers have run into several limitations. For instance, although natural photosynthesis exhibits an almost perfect quantum efficiency (efficient charge separation) [17], it does not in most cases demonstrate a high overall chemical conversion efficiency. In fact, most natural plants can only produce a solar-to-biomass efficiency of about 1% [17, 19, 20], owing to the fact that they have evolved to only be capable of performing enough energy conversion to sustain their own survival [17]. However, it has been determined that the conversion of solar to chemical energy of an industrially feasible artificial photosynthesis system should demonstrate an efficiency of 10% or higher [12, 21]. This has posed as a challenge for this field, being that all of the fabricated devices which have successfully achieved a high conversion efficiency have been made from rare and expensive materials, thus disabling the practicality of scaling up these systems [2].
Furthermore, this ties into the ongoing search for materials that are capable of acting as suitable catalysts for the uphill reactions that artificial photosynthesis entails. Since the processes involved in artificial photosynthesis involve the breaking and forming of chemical bonds, catalysts are necessary to drive this reaction. However, one of the main bottlenecks associated with this area of research is establishing a cost-effective, efficient, and stable catalyst material. A significant issue among studied catalysts such as organic-based is their tendency to be unstable for multiple uses [2, 22]. Many variations have a habit of corroding or degrading the system equipment, while some lose their energy upon several cycles. While plants intrinsically perform self-repair mechanisms, artificial systems most often do not hold this characteristic [19]. Alternative metal-oxide catalysts show good promise, but the ones that have sufficient speed lack abundance and financial viability [2]. Meanwhile, it has been determined that an applicable device should demonstrate stability for at least 10 years [20]. Thus, the search for an appropriate catalyst which exhibits each of these proper functions is ongoing.
Another notable challenge within the area of mimicking a natural process is the complex molecular geometry found in photosynthesizing organisms. Researchers are having a great deal of trouble replicating the level of intricacy that it entails [2]. However, with the help of supramolecular strategies and nanotechnology, scientists are able to easily manipulate the workings of their devices through the structural and molecular composition. Although it is difficult to match the details present in natural photosynthesis, these techniques allow the field to advance toward a viable system [31].
2.3. History
The first proposition of artificial photosynthesis was reported in 1912 by an Italian chemist, Giacomo Ciamician, who acknowledged the unsustainability of fossil fuels. He prompted the idea of replicating nature’s way of producing and storing energy instead [7]. However, no landmark research had been announced regarding this effort until 1972, when Kenichi Honda and his student Akira Fujishima reported the first successful water splitting device powered by light [20], which was named the “Honda-Fujishima effect”. The device entailed a photoelectrochemical cell comprised of a TiO2 photoanode and a platinum (Pt) black cathode, completely submerged in water [21, 22]. When the system was exposed to light, the energy would excite the TiO2, releasing an electron. The “electron hole”, or positive charge, remaining on the Ti atom would be filled by an electron from a water molecule, oxidizing the water to produce oxygen. The released electron would then be donated to a proton derived from water, thus reducing the proton to hydrogen [23]. By irradiating the photoanode with light at wavelengths greater than 400 nm, the device was able to generate oxygen at the photoanode and hydrogen at the photocathode, therefore accomplishing the decomposition of water into oxygen and hydrogen [21] as visualized in figure 3. However, the molecule TiO2 is only capable of absorbing ultraviolet wavelengths, so large amounts of energy could not be extracted in this way [23].
Figure 3. Illustration of water splitting via a photoelectrochemical cell in the “Honda-Fujishima effect” [24]
Later, in 1983, William Ayers from Energy Conversion Devices patented the first visible light water-splitting device. Referred to as an “artificial leaf”, the apparatus was comprised of a thin film multijunction cell made of silicon, with a Nafion membrane for ion transport above the cell, all immersed in water [25]. When illuminated, oxygen was formed on the back metal substrate, while hydrogen evolved on the silicon surface, thus performing photolysis of water into its components [14].
The reduction of CO2 into hydrocarbons driven by solar energy was first achieved in 1978 by M. Halmann [26]. This study used a p-type phosphide semiconductor made of gallium phosphide as the photocathode. The device was suspended in an aqueous solution. When exposed to light, the system successfully produced formic acid (HCOOH), formaldehyde (CH2O), and methanol (CH3OH) [22]. Since these milestones, scientists have dedicated a great amount of research to studying the works of artificial photosynthesis.
3. Artificial Photosynthesis Technicalities
Artificial photosynthesis is a complex objective, assuming many necessary considerations. Overall, the two main functions that need to be completed are the harvesting of light, and the and splitting of water. There is also the possible extension of carbon dioxide fixation for the further production of other fuels besides pure hydrogen [2]. Thus, there are three principal steps in artificial photosynthesis, which are similar to natural photosynthesis: the absorption of light to reach an excited state, charge generation and separation, and chemical conversion for the production of fuel [4].
3.1. Light Absorption
The first step to artificial photosynthesis is the absorption of light photons as a source of energy to drive the system. This zone of research focuses on finding photosensitizers that make optimal use of photon exposure, and are capable of aggregating light energy. One of the limiting characteristics of natural photosynthesis is the fact that most pigment molecules in photosynthesizing organisms can only absorb light at wavelengths within the range of about 400-700 nm [6], which makes up roughly50% of the sunlight that reaches the earth [18], as demonstrated in figure 4.
Figure 4. A visualization of the solar light spectrum available on earth. The green region represents photosynthetically active radiation, which only makes up about half of the sunlight that reaches the earth. [18]
Thus, by implementing materials that are able to make use of a wider utilized band in the solar spectrum, this creates the opportunity to extract a higher amount of applicable energy at a faster rate.
Many systems, inspired by the “Honda-Fujishima effect”, utilize a TiO2 photoanode as a light absorber and catalyst. However, the problem with this material is that it is only capable of absorbing ultraviolet light, so it does not take advantage of the wide spectrum of light available [23]. Moreover, a popular material for semiconductors, as introduced earlier as well, is silicon. Silicon is an attractive material for this purpose because it can absorb a wider spectrum of light, up to 1100 nm [18], and it is also an abundant and cheap source [12]. Other materials that have been studied for this objective include other metal oxides such as ZnO, Fe2O3, and BiVO4, metal nitrides such as Ta3N5, metal phosphides such as GaP, metal oxynitrides such as TaON, etc. [12]. In 2012 Panasonic used a Gallium Nitride semiconductor to produce formic acid and ethanol using thin film technology [42].
An area of interest within this light absorption field is utilizing scientific techniques to manipulate a system and its functions. In this case, several small-scale actions can be taken to induce appropriate light absorption. For instance, elemental doping is very commonly utilized to add specific impurities to semiconductors for the purpose of altering their properties, such as wavelength absorption cutoffs. Furthermore, surface functionalization and rational nanostructuring provide the opportunity to influence a material’s operation. Tweaking the structure of a material is known to be a powerful tool in fine-tuning its purpose. Thus, these strategies aid in doing just that. Under the Blue Sky Project at the University of Michigan, a gallium nitride semiconductor with copper and iron coated silica nanowires has successfully produced methane from CO2 and sunlight [43].
3.2. Water Splitting
Water splitting entails the decomposition of water into oxygen and hydrogen by the means of a chemical redox reaction In one common method, a photoelectrochemical cell incorporates a membrane for separation purposes. Upon irradiation, semiconductor nanowires absorb light and the oxidization of water occurs, producing oxygen, as well as electrons and protons. Those electrons proceed through the wires to the reduction end, while the protons travel there through a proton-conducting membrane, commonly made from Nafion, where protons are reduced to hydrogen. Thus, photolysis of water is achieved through the combination of two different systems, customized for their respective purpose [12].
Figure 5. Side-by-side illustration of a) natural photosynthesis and b) a method of water splitting via artificial photosynthesis by a photoelectrochemical cell with a separation membrane. [12]
The redox equations involved in water splitting can be seen as:
Oxidation: 2H2O → 4e- + 4H+ + O2
Reduction: 4H+ + 4e- → 2H2
Combined Reaction: 2H2O → 2H2 + O2
[21]
Since splitting water requires about 2. 5 V of energy, a catalyst is needed to react with the sunlight photons in order to set off the reaction [2]. As a biomimetic approach, researchers have looked into manganese as a catalytic element because it is found in the photosynthetic core of plants. However, this application often results in instability within an artificial system [14] due to short-term and inefficient function [2]. Furthermore, as mentioned earlier, metal oxides are popular for consideration as catalysts. For instance, a more recently discovered catalyst, cobalt oxide (CoO), is found to be a stable, efficient, and as well as abundant option [2]. In 2019 the artificial leaf was patented using cuprous oxide to produce methanol and 02 from CO2 and sunlight [41].
A notable attribute of water splitting is that it entails the oxidation of water to produce oxygen as well as the reduction of water to produce hydrogen. Many catalysts which function best for one task are insufficient for the other due to their necessary reduction or oxidation potentials [27]. Thus, systems that couple materials which are customized for their respective activity are also considered.
Molecular water-oxidation catalysts are specialized for the evolution of oxygen. Generally, they are transition-metal-based with unsaturated first coordination spheres, and function as active sites for the water molecules, permitting the accumulation of charge to form a high-valent metal-oxo intermediate [28]. For example, ruthenium-based and iridium-based catalysts show good performance due to their reactivity and stability. [12, 19, 28]. However, these elements have the drawback, like many, of being scarce and expensive [12]. Thus, researchers continue to focus on more abundant candidates from the transition metal family such as copper-based, nickel-based, and iron-based [28]. Using molecular techniques to fine-tune compounds containing abundant and redox chemistry-rich metals such as iron in order to improve catalytic function remains a vital objective in this area. Adam Hill at St Lawrence University is creating a Cobalt and Zirconium heterobimetallic combination binuclear unit on a porous silica base which channels energy to a coupled CO2 reducing catalyst. This is combined with a nanotube separation membrane. [40].
Molecular water-reduction catalysts perform the production of hydrogen. The catalysts generally comprise a metal complex with wide open coordination sites and an electronic structure to stabilize a metal-hydride intermediate. Common materials include noble metals such as rhodium-based and platinum-based complexes. However, research mainly focuses on developing catalysts from earth-abundant metals such as cobalt, iron, molybdenum, and nickel. Nickel complexes are reported to be the most stable and efficient hydrogen evolving catalysts out of those listed. Nevertheless, the optimization of material functions through various chemical techniques is still undergoing investigation. [28]
3.3. CO2 Reduction
The reduction, or fixation, of carbon dioxide is another vital process associated with artificial photosynthesis. In addition to the production of oxygen and hydrogen derived from water, there is an interest in generating other hydrocarbon fuels by chemically reducing CO2 with the utilization of hydrogen. Because the carbon atom in CO2 occupies the highest valence, different such fuels can be created depending on the level of reduction [26]. Examples of fuel compounds that can be produced are formic acid (HCOOH), methanol (CH3OH), carbon monoxide (CO) [29], and methane (CH4) [22], for which the reduction reactions can be seen:
CO2 + 2H+ + 2e– → HCOOH [22, 29]
CO2 + 2H+ + 2e– → CO + H2O [22, 29]
CO2 + 6H+ + 6e– → CH3OH + H2O [22, 29]
CO2 + 8H+ + 8e– → CH4 + 2H2O [22]
In contrast with hydrogen fuel, liquid hydrocarbons have the advantage of being easily integrated into our current energy infrastructure, and are therefore a more desirable product [1, 13, 19]. However, generating such hydrocarbon fuels is a greater scientific challenge due to the muli-electron nature of the process, thus imposing additional complexity [13, 19].
Although the exact mechanism has not yet been established, researchers have pieced together an idea of the process that takes place during the reduction of CO2 by light, which is similar to that of water splitting. Thus far, it is believed that CO2 interacts with the catalyst initially, forming an adduct through electrostatic interactions, which triggers the excited photosensitizer to release at least one electron. The electron is transferred to the catalyst, activating the reduction reaction followed by proton coupling. The electron hole in the photosensitizer is immediately filled by an electron donor, and the process is complete [22].
The level of reduction that takes place depends on several elements. The physical and chemical environment plays a large role–that being the temperature, pressure, applied energy, etc. [22]. Although, the largest influence remains the choice of catalyst. Ideal catalyst criteria include a low cost, durable, and selective material. Candidates of research include rhenium-based complexes, as well as more abundant cobalt-based, nickel-based, iron-based, and zinc-based complexes [22, 28].
Although a brilliantly suitable catalyst is yet to be realized, there are several strategies which can be implemented to improve chemical conversion performance. For instance, structural engineering has great influence on the function of a catalyst/system. With the creation of hierarchical porous morphologies, the adsorption of intermediates at the electrode surface could be considerably enhanced [27]. Also, the employment of co-catalysts such as silver, gold, and copper have been found to be an effective approach to boosting conversion efficiency [22]. Many other innovations have been demonstrated for the advancement toward a practical system.
4. Device Designs
There are two prominent devices that have been intricately designed for the purpose of converting solar energy into chemical energy stored in the bonds of a fuel. The first one which will be discussed is the photoelectrochemical cell, and the second one is the photovoltaic-coupled electrolyser. Both systems have similarities and differences to one another, as well as advantages and drawbacks.
4.1. Photoelectrochemical Cell
Photoelectrochemical cells, a main strand of research in this field, consist of two electrodes, a photoanode and a photocathode in contact with an electrolyte and an external wire [13, 22, 28, 30]. Some variations of this system comprise only a single electrode, with the evolution of oxygen and hydrogen as a mixture. However, this version entails contamination and separation issues, adding subsequent complexity, so a two-electrode setup is preferred [31]. The photoelectrodes may be made up of a molecular electrode, or a light-absorbing semiconductor [28]. As explained in the description of the “Honda-Fujishima effect” [23], during photoelectrochemical water splitting, water is oxidized to form oxygen at the photoanode, while it is reduced to form hydrogen at the photocathode [32]. When the cell is exposed to light, solar energy causes excitation in the photoanode, releasing an electron to be donated to a water molecule, thus reducing it to a hydrogen molecule at the photocathode. The “electron hole” remaining on the photoanode would then immediately be filled by an electron from a water molecule, oxidizing water to produce oxygen at the photoanode. A general illustration of this process is demonstrated in figure 6.Figure 6. Schematic illustration of the workings of a common photoelectrochemical cell. [32]
Some photoelectrochemical cell designs also incorporate a membrane as a separation technique. The membrane is most often composed of Nafion, due to its proton-conductive properties [12]. This method of water splitting was also described previously.
The efficiency of a water splitting device depends mainly on the material of the photoanode. Accordingly, the appropriate criteria for a photoanode is high stability in aqueous solution, a wide band gap, and the ability to absorb visible light. Researchers initially used semiconductor materials, but drawbacks occurred such as low efficiency, instability, and a narrow band gap. Thus, focus has shifted toward carbon-based, transition metals, and nanostructured photoanodes. For carbon-based materials, graphene, carbon nanotubes, carbon dots, and carbon quantum dots all demonstrate good stability and photocurrent generation. Transition metals such as germanium doped hematite, cadmium sulfide, zinc oxide, copper sulfide, and molybdenum exhibit excellent performance as well. These materials display outstanding efficiency owing to their high electrical conduction and electrochemical stability. Similarly, nanostructures such as nanowires, nanotapers, and nanorods have been proven to increase hydrogen evolution at the photocathode [32].
4.2. Photovoltaic-Coupled Electrolyser
A photovoltaic-coupled electrolyser incorporates the workings of both a solar cell and an electrochemical cell in separate steps [13, 28, 31]. First, the absorption of light and charge separation is carried out by a photovoltaic cell. Then, the energy potential generated by the cell is applied to an electrolyser to initiate and drive a redox reaction [28]. In this way, solar radiation is initially converted into electricity, which is then used for the oxidation and reduction of water or CO2 in the electrolyser cell [13]. This type of system is considered to be a more efficient approach, for it can achieve solar-to-hydrogen efficiencies of 10-15%, with a record of 30% in 2016 [31]. Figure 7 illustrates a comparison between a photoelectrochemical cell device and a photovoltaic-coupled electrolyser device as well as their potential real-world applications.
Figure 7. Visual representation of a) a photoelectrochemical cell device and its potential application, and b) a photovoltaic-coupled electrolyser and its potential application. [28]
The 2016 ultra-efficient photovoltaic-coupled electrolyser system employed a commercially available triple-junction solar cell. The three subcells were made up of indium gallium phosphide (InGaP), gallium arsenide (GaAs), and gallium indium nitrogen arsenic antimonide GaInNAs(Sb), respectively. The solar cell was connected to two polymer electrolyte membranes in series, which consisted of Nafion membranes. Coating the membranes were a Pt black catalyst at the cathode and a Ir black catalyst at the anode. To begin the process, powered by a current generated in the triple-junction cell, water was pumped into the anode of the first electrolyser. This caused water and oxygen effluent to enter into the anode of the second electrolyser, while hydrogen flowed from the first electrolyser’s cathode to the second electrolyser’s cathode. Hydrogen and oxygen were collected from the cathode and the anode, respectively, at the second electrolyser. Figure 8 illustrates this model. Additionally, unreacted water was sent back into the system to be recycled and used again. The operation continued without interference for 48 hours, and it achieved a remarkable solar-to-hydrogen conversion efficiency of 30%, that being the highest efficiency reported to date. [33]
Figure 8. A photovoltaic-coupled electrolysis system consisting of a triple-junction solar cell connected to two polymer electrolyte membranes in series. [33]
5. Semi-Artificial Photosynthesis
A semi-artificial photosynthesis system actively merges the unique strengths associated with both natural and artificial photosynthesis. For instance, natural photosynthesis offers the valuable advantages of high quantum efficiency (close to 100%), excellent selectivity, and self-repair mechanisms. On the other hand, synthetic approaches provide the ability to implement materials with a wider light absorption spectrum, as well as manipulate molecular chemistries for various purposes. By combining aspects from each end, researchers have the opportunity to controllably engineer the individual components of a system for specific applications, thus minimalizing the drawbacks [17, 34].
5.1. Enzyme Hybrids
Hybridized systems which incorporate synthetic light absorbers with biologically inspired catalysts offer an interesting approach. By driving photocurrents through electrode-wired enzymes, these catalysts can generate kinetically and thermodynamically stubborn products with almost 100% selectivity at fast rates [34].
For example, coupling the photosystem II enzyme with inorganic semiconductors has been studied. In one scenario, isolated photosystem II enzyme complexes were interfaced with a mesoporous and inverse opal indium tin oxide (ITO) electrode. The hierarchical porosity of the electrode featured pores at lengths which match the size of the enzyme. a visual demonstration of this can be seen in figure 9. When paired with a hydrogenase-loaded cathode, the system demonstrated a 5.4% light-to-hydrogen conversion efficiency. Therefore, this strategically connected an inorganic current collector with a biological catalyst to create an advantageous system [34].
Figure 9. Enzyme hybrid system in which photosystem II enzymes are coupled with a synthetic ITO electrode. [34]
5.2. Cell Hybrids
Biohybrid systems which utilize whole-cell implementation have the ability to achieve more complex chemistries in comparison to single-enzyme systems. Cell organisms such as certain types of bacteria can perform high degrees of efficiency and specificity due to their metabolic and enzymatic networks. Thus, the use of inorganic semiconductors or metal nanoparticles interfaced with microorganism cells has acquired its own area of investigation [17].
In one instance, an acetogenic bacterium Moorella thermoacetica were exposed to water-soluble gold nanoclusters AuNCs (mainly Au22(SG)18), where the AuNCs acted as an intercellular photosynthesizer. When the system was illuminated with light at 532 nm, the photogenerated electrons traveled through cytoplasm mediators, bypassing the cell membranes and reaching the bacteria. Meanwhile, the electron holes were filled by cysteine, resulting in its oxidation. The charge transfer ultimately enabled the production of acetic acid from CO2 by the means of the bacteria cells incorporated with biologically-compatible inorganic compounds. The system continued the fixation of CO2 for a longevity of six days. Thus, a whole-cell approach to artificial photosynthesis was successfully carried out [35].
Another approach, incorporating the anaerobe Methanosarcina barkeri, also coupled the biological function of a bacteria species with a materials catalyst. The system occurredtook place in a photoelectrochemical cell made up of nanoparticulate nickel sulfide electrode, a material inspired by naturally occurring nickel-dependent hydrogenases. A culture of bacteria was added to the cathode. When exposed to visible irradiation, the reducing equivalents from electrogenic hydrogen were used to drive the reduction of CO2 to CH4. A diagram of this device can be seen in figure 10. Long-term stability was demonstrated over weeklong7-day electrolysis. During this time, no loss in performance was recorded, and the system only required the restoration of CO2 once every 24 hours. Therefore, the reduction of CO2 into CH4 was executed by the means of a biohybrid system. [36]
Figure 10. A photoelectrochemical cell incorporating the function of Methanosarcina barkeri for the formation of CH4 by CO2 reduction. [36]
6. Strategies for Improvement
Scientists utilize their knowledge of nanotechnology and molecular manipulation to implement strategies which can aid in various purposes. For example, elemental doping is one technique used to add impurities to semiconductors which can alter their function. Functions that are desirable include a wide light absorption capability, efficient catalyst performance, and selectivity [13].
Moreover, supramolecular strategies are commonly used when dealing with the construction of devices based on molecular components. Organization of chromophores can lead to energy funneling and charge separation at faster rates. In the case of catalyst optimization, catalysis can be enhanced by supramolecular preorganization, and stability can be reinforced by supramolecular cages which prevent degradation. Therefore, the ability to maneuver specific functions in this way is a significant advantage of molecular systems. [31]
To continue, the development of different cell configurations and structures plays an important role in the functionality of a device. For instance, a single-photoelectrode in comparison with a tandem setup can determine great differentiation. Similarly, photoelectrochemical cells show separate performance from a photovoltaic-coupled electrolyser [30]. Furthermore, rational nanostructuring can provide the opportunity to influence a material’s operation by the way the surface is functionalized. Minor tuning to a material’s structure can influence its mechanism a great deal [13].
Finally, operative conditions also help to influence the workings of a chemical system. Specifically, the temperature, pressure, and ion concentration of the environment hold significant leeway. Changing these conditions can result in completely different outcomes for the same setup. In many cases, tweaking the temperature or pressure of a system can conclude in a much more operative effect [30].
7. Future Impact and Concluding Remarks
Just as the study of bird flight influenced the engineering of airplanes, natural photosynthesis serves as humanity’s guide to mimic the functions of a self-sustaining organism, to hopefully one day facilitate a self-sustaining world. The search for a renewable source of energythat can relieve society’s current state of crisis is a top priority for many scientists and engineers. With quickly depleting fossil fuels as our main supply, the worldwide energy consumption is anticipated to increase by more than 50% by the mid-2000s [28]. Thus, the need to mitigate this issue is significant, and the world of scientific research recognizes that.Although groundbreaking research has been conducted throughout this field, the endeavor toward a viable system is still in its infancy. At this point, researchers have achieved many successful versions of artificial photosynthesis. However, all of the methods proposed have suffered from drawbacks of either inadequate efficiency/speed, long-term instability, or financial expense [6]. Natural photosynthesis took billions of years for evolution to develop, and humanity is attempting to master it in decades. Experts anticipate that this type of system will not be ready for industrial utilization for at least ten more years [2].
Nevertheless, the search for a cost-effective, robust, and scalable system continues. Researchers working with The Liquid Sunlight Alliance (LiSA) and The Center for Hybrid Approaches in Solar Energy to Liquid Fuels (CHASE) are using the $100 million funding granted by the U.S. Department of Energy to crack down on this challenge, and soon develop a technology that is ready for large-scale application. Furthermore, the U.S. is not the only country focusing on this topic. This type of research is heavily studied in different parts of the world such as China, Japan, and the European Union [4]. Figure 11 shows a representation of the number of papers that have been published on artificial photosynthesis in several different countries.
Figure 11. Representation of the number of publications on artificial photosynthesis in each country to date in 2019. [4]
Artificial photosynthesis could provide an efficient and sustainable system of generating, storing, and transporting energy. For instance, it can be used to produce hydrocarbon fuels, which can act as beneficial substitute for fossil fuels. It can also produce hydrogen fuel, which can be applied in a number of ways. Channeling hydrogen produced by photolysis into a fuel cell can be used to generate electricity [18]. Figure 12 illustrates a proposed concept of solar energy production, storage, and application.
Figure 12. An illustrative proposition of a future means of producing hydrogen as a storable solar fuel and its application for electricity generation. [18]
Moreover, just as solar panels can be installed onto roofs, providing a secondary source of electricity, future artificial photosynthesis devices can also be applied to power homes. Instead, this system offers a way to store the energy for later use.
Furthermore, hydrogen-powered cars have emerged as an option in the world of transportation. More than 60% of global oil depletion is used for transport [18]. Thus, electric cars are becoming increasingly popular , and models such as the Toyota Mirai [37], the Hyundai Nexo [38], and the Honda Clarity [39] are now powered by hydrogen, which can be sufficiently produced by artificial photosynthesis Hydrogen-powered vehicles claim several advantages within the electric vehicle industry. For instance, they require a short refueling time of around three minutes, are able to last a distance on one full charge which matches that of a gasoline-powered car, unlike most electric vehicles which can take hours of charging time and run out of fuel quickly [44, 45]. Additionally, in terms of environmental consciousness, hydrogen-powered cars emit zero harmful byproducts, which is more than gasoline-powered cars can claim [44].
Furthermore, a system which uses artificial photosynthesis to produce hydrogen for vehicle fuel is hypothetically practical. To put things into perspective, four moles of sunlight photons are required to generate one mole of hydrogen. The surface of the earth is exposed to an average of 10-120 moles of photons for every square meter in a single day. This means that between 2.5 to 30 moles of hydrogen could be produced per square meter every day. That equates to about 5-60 grams of hydrogen per square meter per day, and the Honda Clarity uses about 500 grams of hydrogen per day to run. Thus, only 10-20 square meters, which is about the area of a garage roof, would be needed to fuel a car. [18]
Artificial photosynthesis is the key to mimicking nature’s most effective way of producing energy rich fuel from abundant, renewable inputs. Powering society with chemically converted natural resources like water and carbon dioxide is ideal for our future. In an optimized system, an artificial photosynthesis device could be designed to extract excess CO2 from the environment, all while releasing oxygen back into it. Therefore, not only would this method be a way to generate clean fuel with no harmful byproducts, but it would also contribute toward reversing global warming [2]. Although the effort is burdensome, the concept of renewable energy by artificial photosynthesis is a valuable and promising solution.
About the Authors:
Dr. Raj Shah is a Director at Koehler Instrument Company in New York, where he has worked for the last 25 years. He is an elected Fellow by his peers at IChemE, CMI, STLE, AIC, NLGI, INSTMC, The Energy Institute and The Royal Society of Chemistry An ASTM Eagle award recipient, Dr. Shah recently coedited the bestseller, “Fuels and Lubricants handbook”, details of which are available at https://www.astm.org/DIGITAL_LIBRARY/MNL/SOURCE_PAGES/MNL372ND_foreword.pdf
A Ph.D in Chemical Engineering from The Penn State University and a Fellow from The Chartered Management Institute, London, Dr. Shah is also a Chartered Scientist with the Science Council, a Chartered Petroleum Engineer with the Energy Institute and a Chartered Engineer with the Engineering council, UK. An adjunct professor at the Dept. of Material Science and Chemical Engineering at State University of New York, Stony Brook, Raj has over 350 publications and has been active in the alternative energy field for 3 decades.
More information on Raj can be found at
Ms. Eliana Matsil is part of a thriving internship program at Koehler Instrument company and a student at State University of New York, Stony Brook, where Dr. Shah currently heads the External advisory board of directors at the Dept. of Chemical Engineering.
Ms. Gabrielle Massoud has a BS in Chemical engineering and an MS in biomedical engineering. she has over 20 plus yrs experience in the energy sector and has previously worked for ExxonMobil and Soltex, inc. Lately she has been working in the field of alternative energy, biopolymers and is active in the area of finding sustainable solutions for our planet.
Reference
[1] Styring S. Artificial photosynthesis for solar fuels. Faraday Discuss. 2012;155:357–76.
[2] Layton J. How Artificial Photosynthesis Works [Internet]. HowStuffWorks Science. HowStuffWorks; 2020 [cited 2021Jan31]. Available from: https://science.howstuffworks.com/environmental/green-tech/energy-production/artificial-photosynthesis.htm
[3] Purchase R, De Vriend H, De Groot H. Harmsen P, Bos H, editors. Artificial Photosynthesis For the conversion of sunlight to fuel. 2015Dec;
[4] Durrant J. Artificial Photosynthesis - Concluding Remarks. Faraday Discussions. 2019Jun5;
[5] Bennett2009-04-28T14:22:16+01:00 H. The artificial leaf [Internet]. Chemistry World. 2009 [cited 2021Jan31]. Available from: https://www.chemistryworld.com/features/the-artificial-leaf/3004813.article
[6] Artificial Photosynthesis [Internet]. TheGreenAge. 2017 [cited 2021Jan31]. Available from: https://www.thegreenage.co.uk/tech/artificial-photosynthesis/
[7] Davey T. Artificial Photosynthesis: Can We Harness the Energy of the Sun as Well as Plants? [Internet]. Future of Life Institute. Tucker Davey https://futureoflife.org/wp-content/uploads/2015/10/FLI_logo-1.png; 2018 [cited 2021Jan31]. Available from: https://futureoflife.org/2016/09/30/artificial-photosynthesis/
[8] Department of Energy Announces $100 Million for Artificial Photosynthesis Research [Internet]. Energy.gov. [cited 2021Jan31]. Available from: https://www.energy.gov/articles/department-energy-announces-100-million-artificial-photosynthesis-research
[9] Basics of Photosynthesis | PRO-MIX Greenhouse Growing [Internet]. [cited 2021Jan31]. Available from: https://www.pthorticulture.com/en/training-center/basics-of-photosynthesis/
[10] Vidyasagar A. What Is Photosynthesis? [Internet]. LiveScience. Purch; 2018 [cited 2021Jan31]. Available from: https://www.livescience.com/51720-photosynthesis.html
[11] Andreiadis ES, Chavarot-Kerlidou M, Fontecave M, Artero V. Artificial Photosynthesis: From Molecular Catalysts for Light-driven Water Splitting to Photoelectrochemical Cells. Photochemistry and Photobiology. 2011;87(5):946–64.
[12] Poudyal RS, Tiwari I, Koirala AR, Masukawa H, Inoue K, Tomo T, et al. Hydrogen production using photobiological methods. Compendium of Hydrogen Energy. 2015;:289–317.
[13] Barber J, Tran PD. From natural to artificial photosynthesis. Journal of The Royal Society Interfac
This post does not have any comments. Be the first to leave a comment below.The content & opinions in this article are the author’s and do not necessarily represent the views of AltEnergyMag
Comments (0)
Featured Product
Quality assurance in the manufacturing industry
To continue without subscribing, click the Close button or hit the ESC key.
Please Take a Moment to Subscribe to our Newsletter
To continue without subscribing, click the Close button or hit the ESC key.
© 2010 - 2025 AltEnergyMag - All Rights Reserved
Powered by BTH Management